Part One was the Introduction to The Highs & Lows of Altitude
In Part Two we will discuss… Oxygen Dynamics - The mechanisms behind the uptake, transport and delivery of oxygen at sea level and altitude.
Before we get into the nitty-gritty of the practice of altitude training, I will explain:
the physiology of how oxygen gets from the outside air into our muscle cells
how exposure to altitude results in adaptations which improve the efficiency of the oxygen processing mechanisms to create more energy
Understanding how the body processes oxygen enables better targeting of altitude training. There is a mountain of scientific literature on the topic through textbooks and articles. The scientific nature of the information makes it more difficult to understand.
This article aims at coaches and athletes who do not have a science background. I have translated the scientific information regarding oxygen dynamics into more straightforward and practical terms to help understand how our body adapts to the reduced availability of oxygen at altitude and how these adaptations help improve energy availability when returning to sea level.
Oxygen is the component of air which links us to life. It plays an essential role in creating energy out of carbohydrates and fat which we ingest and store in our bodies. This energy is used to maintain autonomic bodily functions and, when extra energy is required, for specific tasks, including exercise. The muscles can achieve more work when there is more energy available. The maximum amount of oxygen we can take up and process (VO2 max) is a powerful predictor of endurance performance, and the most effective way of improving our VO2 max is through physical training (improvements of up to twenty per cent). Our VO2 max potential and our ability to improve it are, for the most part, genetically determined. One reported outcome of altitude training is an additional improvement of VO2 max up to 7%.
Interestingly there is also evidence of performance improvements following altitude training, without an accompanying increase in VO2 max. Indicating there must be other adaptations besides an increase in VO2 max, which can contribute to improved oxygen utilisation in the metabolic process to create energy.
The Oxygen Pathway
The air which surrounds us and which we inhale is called ambient air. Air consists of 78% of nitrogen (N2) and 21% oxygen (O2). The remaining 1% comprises small amounts of carbon dioxide (CO2), argon (Ar) and helium (He).
Air is subject to the earth's gravitational forces, which give it a specific density (the amount of oxygen and nitrogen molecules in a shared space), translating into pressure. The denser the air is, the higher the pressure it exerts. The (barometric) pressure of ambient air at sea level, where the gravitational forces are strongest, and the air, therefore, most dense, is 760mmhg. The partial pressure of oxygen (PO2) in the air is the contribution of oxygen pressure to the total pressure of the air. As oxygen makes up 21% of the air at sea level, the pressure contributed by oxygen is 21% of 760mmhg, which is 159mmhg.
Air is inhaled deep inside the lung into little vesicles, called alveoli, where the oxygen filters into the circulation. The lungs contain some 300 million alveoli. The blood is pumped to the lung by the right side of the heart via the pulmonary artery. When oxygen transfers from the lung into the circulation, most of it attaches to haemoglobin. Haemoglobin is an oxygen-carrying protein present within the red blood cells. Pulmonary circulation exits the lungs via the pulmonary veins, which transport the oxygen-enriched blood to the left heart (the pulmonary veins are the only veins in the body that carry oxygen-rich blood). The heart subsequently pumps the oxygen-rich blood to the body's organs via the main artery, the aorta and its branches (Figure 1).
Once the blood reaches its target organ, oxygen is released from haemoglobin to enter the cells. The final step is the transport of oxygen from the cell fluid into the mitochondria. The mitochondria are the energy-producing factories within each cell. The target organs for oxygen include all body tissues, including the brain, liver, kidneys, gut, muscles, and other organs. Once oxygen arrives in the mitochondria it aids in metabolising the two primary energy substrates, carbohydrates and fat, to create the energy which supports the various bodily functions. For this text, we will focus mainly on the muscle as the target organ for oxygen. The muscle cell uses the energy for muscle contraction, which generates movement. Increasing oxygen availability results in more energy production to support contractile forces. For endurance athletes, increased oxygen availability for the muscles translates into an ability to last longer at a sustained higher intensity pace, subject to ongoing availability of carbohydrate and fat energy substrates. The improvements in oxygen uptake and utilisation, achieved through physical training, can be further enhanced by the adaptations of the oxygen pathway, achieved through depriving the body of its full quota of oxygen, when residing and training at altitude.
The Oxygen Cascade
The oxygen pathway from the outside air into the mitochondria is called the oxygen cascade. The reason for this is that there is a significant pressure difference between the partial pressure of oxygen (PO2) in the ambient air (159mmhg) and the PO2 in the mitochondria (10-20mmhg). This considerable pressure gradient is the main force behind the oxygen flow from the outside air into the lungs, from the lungs into the circulation and from the circulation into the muscle cell and, finally, the mitochondria, as any fluid or gas substance will automatically "cascade" down from a high pressure (high concentration) area to an area with lower pressures (low concentration). This process is called diffusion (Figure 2).
The cells don’t absorb all the oxygen the arteries transport to the body tissues. Besides containing some residual oxygen, the blood which returns via the right heart to the lungs, transports by-products (CO2, H2O, lactate and protons) from the metabolic process. In the lungs, CO2 is exchanged for oxygen. The liver and other body organs recycle or deactivate the other by-products. Of these, lactate is recycled as a (very efficient) fuel. All parts of the oxygen pathway are essential and contribute to the one integrated oxygen uptake, transport and utilisation system.
Besides the pressure gradient, some other processes help facilitate oxygen transport to the mitochondria. The following outlines the details of the exact mechanisms behind the oxygen cascade:
Step 1: Diffusion of oxygen from the ambient air into the alveoli.
The PO2 of ambient air at sea level is 159mmhg, and the PO2 deep in the alveoli is 100mmhg. The reason for the PO2 difference between ambient air (159mmhg) and the air in the alveoli (100mmhg) is the fact that water vapour is added to the air (replacing some of the oxygen) on inhalation when the air is wetted and warmed by the lining of the upper airways. In addition, oxygen is continuously extracted from the alveolar air into the circulation in exchange for carbon dioxide. The water vapour effect, combined with mixing the old and fresh air, results in a total PO2 gradient between the outside air and the air in the alveoli of almost 60mmhg. Active inhalation aids the oxygen diffusion from the outside air deep into the lungs.
Step 2: Diffusion of oxygen from the alveoli into the circulation.
The right side of the heart pumps blood with a low oxygen content (PO2 of 40mmhg) to the circulation of the lungs via the pulmonary artery (the only artery in the body containing oxygen-poor blood). As the PO2 within the alveoli is 100mmhg, oxygen will rapidly cascade into the bloodstream until the PO2 in the pulmonary circulation equals the PO2 of the alveoli. At rest, this process is completed well before the circulation exits the lungs via the pulmonary vein.
Step 3: Transport of oxygen via the circulation to its target organ.
Once the oxygen molecules enter the microcirculation of the lungs, the majority attach themselves quickly to the haemoglobin, a protein within the red blood cells, which transports the oxygen to the cells of the target organs. A small amount of oxygen (approximately 3%) travels freely, dissolved and unattached in the blood. This 'free' oxygen is responsible for the level of PO2 in the blood. Once attached to haemoglobin, oxygen loses its capacity to exert pressure. The PO2 in the blood is closely related to the number of haemoglobin receptors occupied by oxygen, also called arterial oxygen saturation (SaO2). A pulse oximeter is an electronic device that measures the SaO2 of the red blood cells. Pulse oximeters can be attached to your fingers (Figure 3). This simple device is a must for every athlete who trains at altitude to monitor the level of 'hypoxic' stress they are under. The SaO2 measured by way of a pulse oximeter is called SpO2.
Step 4: Oxygen diffusion from the arterial circulation into the muscle cell.
On arrival at the cells of the target organ, oxygen off-loads from haemoglobin with the help of an enzyme called 2,3-DPG (2,3-diphosphoglycerate). The PO2 in the circulation is well preserved between the time it exits the lung (PO2 100mmhg) and arrives at the cells of the target organ (PO2 95-98mmhg) under sea-level resting conditions. At rest, within the cytoplasm, the fluid within the muscle cell, the PO2 is approximately 30mmhg. This significant pressure gradient allows for a relatively easy oxygen flux from the circulation into the muscle cell once oxygen is released from haemoglobin.
Step 5: Transport from the cytoplasm (cell fluid) to the mitochondria.
Once inside the muscle cell, oxygen is bound to a transport protein called myoglobin which carries it to the mitochondria.
Step 6: Diffusion of oxygen from the cytoplasm into the mitochondria.
Oxygen pressure within the mitochondria at rest fluctuates between 10 and 20mmhg. The 15mmhg or so pressure difference between the cell fluid and the mitochondria allows the oxygen to diffuse one more time before it can execute its role in the metabolic process to generate energy via the creation of adenosine triphosphate (ATP).
The Oxygen Cascade During Exercise
Exercise changes the dynamics of the oxygen cascade. More fuel (oxygen) is necessary to produce the energy which drives muscle contraction. During high-intensity exercise, the PO2 in the mitochondria drops quickly to 2-5mmhg due to increased oxygen usage. There is an immediate response from the lungs and circulation in an attempt to meet this increased oxygen demand by the mitochondria. In well-trained individuals, ventilation increases dramatically from about 6 litres to up to 200 litres of air per minute. Not all the air reaches the alveoli, but a significant amount does, increasing alveolar PO2 from 100mmhg to 120mmhg, which improves the PO2 gradient between the lungs and the circulation. A slight drop in PO2 in the circulation, presented to the lungs via the pulmonary artery (PO2 drops from 40mmhg to 35-38mmhg), further enhances the pressure gradient between the lungs and the circulation. Due to the increased PO2 gradient, oxygen diffuses more rapidly from the alveoli into the bloodstream.
The circulation also speeds up through a significant increase in cardiac output. The cardiac output is the blood volume pumped by the heart's left chamber into the circulation per minute. Our heart rate (beats per minute) and stroke volume (the amount of blood pumped out by each contraction) control the cardiac output (cardiac output=stroke volume X heart rate). At rest, the cardiac output is approximately 5-6 litres per minute. During high-intensity exercise in very fit athletes, this can increase 8-fold to 40 litres per minute. The increase in circulation can thus accommodate the rise in oxygen flux from the lung into the blood. A trained athlete has a superior ability to process oxygen downstream at cellular level and demand for oxygen can outstrip supply at high intensities. This causes a drop in PO2 (and a related decrease in SpO2) in the arterial circulation as there is a relative limitation to the oxygen diffusion capacity of the lungs. For optimal energy production, the PO2 in the mitochondria needs to be at least 2mmhg. When it drops below 2mmhg, the metabolic process is compromised, and less energy is produced, resulting in a drop in performance.
The Oxygen Cascade At Altitude
At altitude, further away from the forces of gravity, the air has a lower pressure and is less dense than at sea level. The higher you go, the less dense the air and the lower the air pressure. The lower air density at altitude means that the nitrogen and oxygen molecules, which make up the air, are further apart, resulting in a lower amount of oxygen in a given amount of air compared to sea level (Figure 4).
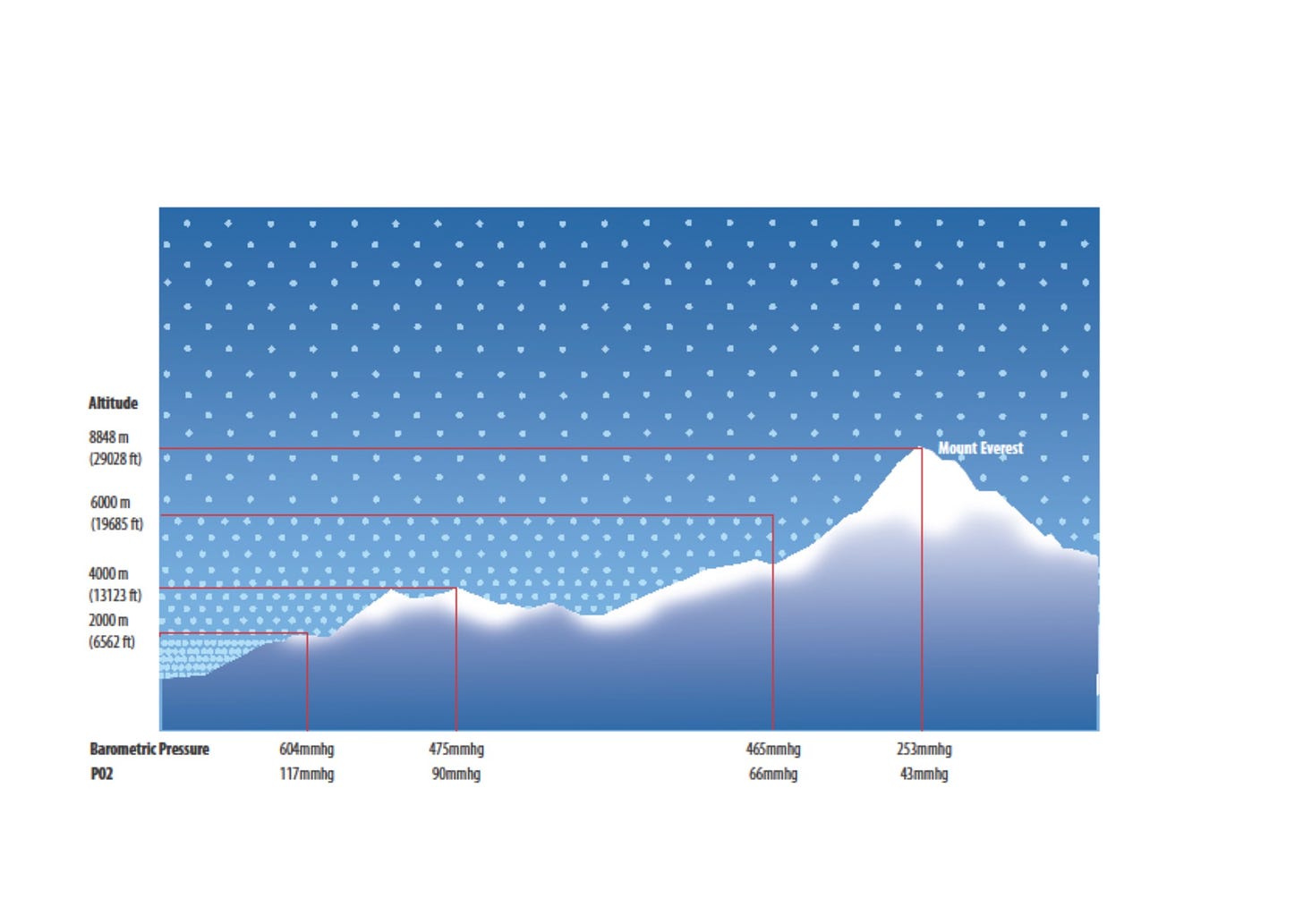
At altitude, a similar oxygen cascade occurs as at sea level, except the starting PO2 in the outside air is lower. This immediately affects the PO2 further down the cascade. Increasing altitude diminishes the total pressure gradient between the ambient air and mitochondria. Flattening the pressure gradient between the outside air and the mitochondria threatens the oxygen delivery process, and the body jumps into action by compensatory mechanisms.
In the next article, I will explain the adaptations that occur at altitude and improve oxygen processing mechanisms. I will also explore how different altitudes affect our bodies and why there is an optimal altitude zone for us to live and train to achieve our desired results.
Back To Table of Contents
References & Further Reading
P D Wagner. Systemic Oxygen Transport and Utilisation. Topical Review. J Breath Res. 2 (2008)
John B West, Robert B Schoene and James S Milledge. High Altitude Medicine and Physiology. Fourth Edition
Robert S Mazzeo. Physiological Responses to Exercise at Altitude. Sports Med 2008; 38 (1): 1-8
Christopher John Gore, Sally A. Clark and Philo U Saunders. Nonhematological Mechanisms of Improved Sea-Level Performance after Hypoxic Exposure. Med Sci Sports Ex, 2007…..
Lawrence Martin, MD. All You Need to Know to Interpret Arterial Blood Gases. Second Edition